- Part I
Vaccinology before Covid - Vaccinology
- A brief history of vaccination
- Vaccines; in general
- Antigens, Epitopes and Antibodies
- Antigens
- Epitopes
- Antibodies (Abs)
- Antibody structure
- Antibody subclasses
- Vaccines types; in general
- Vaccines induce antibodies
- Immunodeficiency states
- Passive protection
- Immunological data
- Vaccines need T-cell help
- Vaccine induced protection
- Immune memory
- Herd immunity
- Factors affecting vaccine effectiveness
- Reverse Vaccinology (RV)
- Omicron and those already vaccinated
- Vaccine-mediated immune preconditioning
- Hybrid immune damping
Vaccinology, Vaccines, Vaccination & Covid-19
Part I
Vaccinology before the Covid-19 pandemic
Vaccinology
Over the last two decades, a new field of microbiology and immunology has evolved called “vaccinology” that comprises not only vaccine development but also the use of vaccines and their effects on public health.
Vaccines exploit the extraordinary ability of the highly evolved human immune system to respond to, and remember, encounters with antigens. Throughout much of human history, vaccines have been developed through empirical research and observation.
Because the SARS-CoV-2 coronavirus seems to behave differently than other viral pathogens which have ravaged the human species throughout history, this article must necessarily be divided into two parts: Vaccinology based upon the body of immunological literature before the SARS-CoV-2 coronavirus and vaccinology since the SARS-CoV-2 coronavirus pandemic began.
In Part I, much of the following material has been adapted from: Pollard, A.J., Bijker, “E.M. A guide to vaccinology: from basic principles to new developments.” Nat Rev Immunol 21, 83–100 (2021). https://doi.org/10.1038/s41577-020-00479-7 and augmented by the author of this page.
A brief history of vaccination
Epidemics of smallpox swept across Europe in the seventeenth and eighteenth centuries, accounting for as much as 29% of the death rate of children in London. Initial efforts to control the disease led to the practice of variolation, which was introduced to England by Lady Mary Wortley Montagu in 1722, having been used in the Far East since the mid-1500s (see Nature Milestones in Vaccines). In variolation, material from the scabs of smallpox lesions was scratched into the skin in an attempt to provide protection against the disease. Variolation did seem to induce protection, reducing the attack rate during epidemics, but sadly some of those who were variolated developed the disease and sometimes even died. It was in this context that Edward Jenner wrote “An Inquiry into the Causes and Effects of the Variole Vaccinae…” in 1798. His demonstration, undertaken by scratching material from cowpox lesions taken from the hands of a milkmaid, Sarah Nelms, into the skin of an 8-year-old boy, James Phipps, who he subsequently challenged with smallpox, provided early evidence that vaccination could work. Jenner’s contribution to medicine was thus not the technique of inoculation but his startling observation that milkmaids who had had mild cowpox infections did not contract smallpox, and the serendipitous assumption that material from cowpox lesions might immunize against smallpox. Furthermore, Jenner brilliantly predicted that vaccination could lead to the eradication of smallpox; in 1980, the World Health Assembly declared the world free of naturally occurring smallpox.
Almost 100 years after Jenner, the work of Louis Pasteur on rabies vaccine in the 1880s heralded the beginning of a frenetic period of development of new vaccines, so that by the middle of the twentieth century, vaccines for many different diseases including diphtheria, pertussis and typhoid had been developed as inactivated pathogen products or toxoid vaccines. However, it was the coordination of immunization as a major public health tool from the 1950s onwards that led to the introduction of comprehensive vaccine programs.
Vaccines; in general
The essential component of most vaccines is one or more protein antigens that induce immune responses which will provide protection. However, polysaccharide antigens can also induce protective immune responses and are the basis of vaccines that have been developed to prevent several bacterial infections, such as pneumonia and meningitis caused by Streptococcus pneumoniae, since the late 1980s.
Protection conferred by a vaccine is measured in clinical trials that relate immune responses to the vaccine antigen to clinical end points such as prevention of infection, a reduction in disease severity or a decreased rate of hospitalization.
Vaccines are generally classified as live or non-live which are sometimes loosely referred to as “inactivated” to distinguish those vaccines that contain attenuated replicating strains of the relevant pathogenic organism from those that contain only components of a pathogen or killed whole organisms.
Antigens, Epitopes and Antibodies
During the first half of the 20th century, a series of scientific discoveries resolved that antibody-mediated immunity is the cornerstone of the specific immune response. A specific antibody will combine with its specific antigen to generate an exclusive antibody-antigen complex.
Protection conferred by a vaccine is measured in clinical trials that relate immune responses to the vaccine antigen to clinical end points such as prevention of infection, a reduction in disease severity or a decreased rate of hospitalization.
Antigens
The term antigen is derived from antibody generation, referring to any substance that is capable of eliciting an immune response such as the production of specific antibody molecules. By definition, an antigen (Ag) is capable of combining with the specific antibodies formed by its presence.
Generally, antigens are foreign proteins or their fragments that enter a host body through an infection. However, in some cases, the body’s own proteins may act as antigens and induce an autoimmune response. Bacteria and viruses contain antigens, either on their surface, or inside. These antigens can be isolated and used to develop vaccines.
Antigens are generally of high molecular weight, and commonly are proteins or polysaccharides, however, polypeptides, lipids, nucleic acids, and many other materials can also function as antigens. Immune responses may also be generated against smaller substances, called haptens, if these are chemically coupled to a larger carrier protein, such as bovine serum albumin, keyhole limpet hemocyanin (KLH), or other synthetic matrices. A variety of molecules such as drugs, simple sugars, amino acids, small peptides, phospholipids, or triglycerides may also function as haptens.
Given enough time, just about any foreign substance will be identified by the immune system and evoke specific antibody production. However, this specific immune response is highly variable and depends much in part on the size, structure, and composition of antigens. Proteins or glycoproteins are considered the most suitable antigens due to their ability to generate a strong immune response. They are strongly immunogenic.
Antigens are recognized by the host body through two distinct processes (1) by B cells and their surface antibodies (sIgM) and (2) by the T cell receptor on T cells. Although both B and T cells respond to the same antigen, they respond to different parts of the same molecule. Antibodies on the surface of B cells can recognize the tertiary structure of proteins; T-cells require antigens that have been ingested and degraded into recognizable fragments by the antigen-presenting cells. Commonly employed antigen-presenting cells are macrophages and dendritic cells.
The immune response
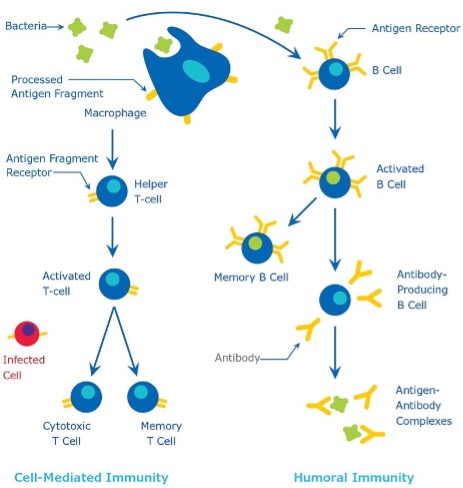
The immune response
Epitopes
The small site on an antigen to which a complementary antibody may specifically bind is called an epitope or antigenic determinant . This is usually one to six monosaccharides or five to eight amino acid residues on the surface of the antigen. Because antigen molecules exist in space, the epitope recognized by an antibody may be dependent upon the presence of a specific three-dimensional antigenic conformation; a unique site formed by the interaction of two native protein loops or subunits. This is known as a conformational epitope. The epitope may also correspond to a simple linear sequence of amino acids and such epitopes are known as linear epitopes.
The range of possible binding sites on a target molecule (antigen) is enormous, with each potential binding site having its own structural properties derived from covalent bonds, ionic bonds, hydrophilic, and hydrophobic interactions. For efficient interaction to occur between the target antigen and the antibody, the epitope must be readily available for binding.
If the target molecule is denatured, e.g., through fixation, reduction, or pH changes, the epitope may be altered and this may affect its ability to interact with an antibody.
In their natural form, antigens may be cytoplasmic (soluble), membrane-associated, or secreted. The number, location and size of the epitopes depend on how much of the antigen is presented during the antibody-making process.
Amino acids forming a protein0
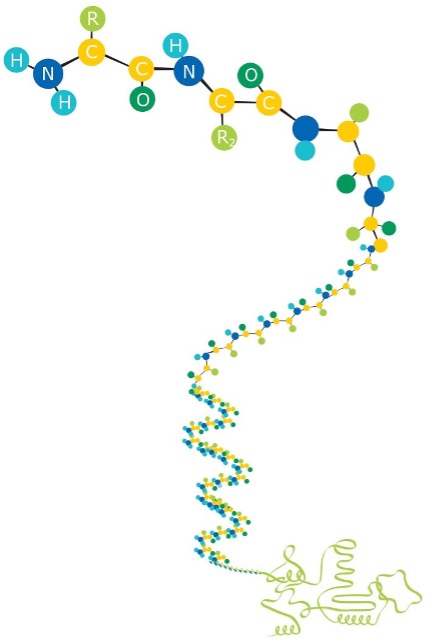
Amino acids forming a protein
Antibodies (Abs)
An antibody is defined as “an immunoglobulin capable of specific combination with the antigen that caused its production in a susceptible animal.” Antibodies are produced in response to the invasion of foreign molecules in the body. An antibody, abbreviated as Ab, is commonly referred to as an immunoglobulin or Ig. Human immunoglobulins are a group of structurally and functionally similar glycoproteins (82-96% protein and 4-18% carbohydrate) that confer humoral immunity.
Antibody structure
Antibodies exist as one or more copies of a Y-shaped unit, composed of four polypeptide chains. Each Y contains two identical copies of a heavy chain and two identical copies of a light chain, named as such by their relative molecular weights. This Y-shaped unit is composed of the two variable, antigen-specific F(ab) arms, which are critical for actual antigen binding, and the constant Fc “tail” that binds immune cell Fc receptors and also serves as a useful “handle” for manipulating the antibody during most immunochemical procedures. The number of F(ab) regions on the antibody corresponds with its subclass (see below), and determines the valency of the antibody (loosely stated, the number of “arms” with which the antibody may bind its antigen).
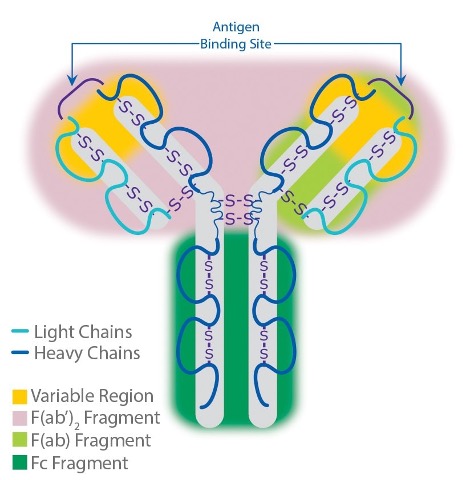
Antibody structure
Antibody subclasses
Antibodies can be divided into five classes: IgG, IgM, IgA, IgD, and IgE, based on the number of Y units and the type of heavy chain. Heavy chains of IgG, IgM, IgA, IgD, and IgE, are known as g, µ, a, d, and e, respectively. The light chains of any antibody can be classified as either a kappa (κ) or lambda (λ) type (based on small polypeptide structural differences); however, the heavy chain determines the subclass of each antibody.
The subclasses of antibodies differ in the number of disulfide bonds and the length of the hinge region. The IgG class is the major immunoglobulin class released in serum.
IgA: In the blood IgA are present in low levels in monomeric form. They are most active at mucosal surfaces where they are present in dimeric form and provide the primary defense at mucosal surfaces. More IgA is produced in mucosal linings than all other types of antibody combined. Its major function is to act as a neutralizing antibody. High levels of IgA are present in saliva, tears, and breast milk. In humans two IgA subtypes are known to exist. IgA1 may account up to 85% of the total IgA in serum. Selective IgA deficiency is one of the most common immunodeficiency diseases that increases susceptibility to infections. IgA deficiencies are commonly seen in patients with autoimmune diseases and allergic disorders. IgA has a half-life of about 5 days.
IgD: It is a monomeric antibody with two epitope binding sites and is found on the surface of most B lymphocytes. Its precise function is still disputed, but is suggested to acts as an antigen receptor required for B cell activation. IgD is also reported to bind to basophils and mast cells and activate them to produce antimicrobial factors. It’s also believed to play a role in eliminating B-lymphocytes that produce self-reactive autoantibodies. IgD is also produced in a secreted form that is found in serum in small quantities and contains two heavy chains of the δ class and two light chains. IgD has a half life of about 3 days.
IgE: This group of antibodies is effective at mucosal surfaces, blood, and tissues. It is present as monomer consisting of two heavy chains (ε chain) and two light chains. The ε chain contains 4 Ig-like constant domains. In serum, it is present in low concentrations contributing to only about 0.002% of total serum antibodies. Most IgE is tightly bound to its receptors on mast cells and basophils via the Fc region. It plays a crucial role in hypersensitivity reactions and its production is strictly controlled by cytokines. IgE has a half-life of about 2 days.
IgG: This is the most abundant class of antibodies in the blood, comprising up to 80% of the total serum antibodies. It is present in monomeric form. Four subclasses of IgG have been described depending on their abundance (IgG1>IgG2>IgG3>IgG4) and the subclass produced is dependent on the type of cytokine present.
IgG1 and IgG3 exhibit high affinity for Fc receptors on phagocytes, while IgG2 exhibits very low affinity and IgG4 has moderate affinity for Fc receptors IgGs are capable of exiting the circulatory system and enter tissues. IgG1, IgG3, and IgG4 can cross placental barrier to provide protection for newborns. IgGs are efficient at activating the complement system, and are very effective for opsonization using Fc receptors on phagocytes. Through its Fc region IgG can also bind to natural killer cells and participate in antibody-dependent cytotoxicity. IgG has a half-life ranging from 7 to 23 days, depending on its subclass.
IgM: This class of immunoglobulin is first to be produced in response to infection and is found either on membranes of B cells or as a 5-subunit macromolecule secreted by plasma cells. It is also the first immunoglobulin class to be synthesized by neonates. The surface IgM differs from the secreted form in its Fc region. Surface IgM binds directly as an integral membrane protein and not to the IgM Fc receptor. Secreted IgM is a pentameric molecule where multiple immunoglobulins are covalently linked with disulfide bonds. This structure provides multiple binding sites. Each monomer consists of two light chains (either κ or λ) and two heavy chains. Because of its pentameric nature IgM is particularly suited for activating complement and causing agglutination. IgM has a half-life of about 5 days.
Vaccine types; in general
Vaccines are generally classified as live or non-live which are sometimes loosely referred to as “inactivated” to distinguish those vaccines that contain attenuated replicating strains of the relevant pathogenic organism from those that contain only components of a pathogen or killed whole organisms.
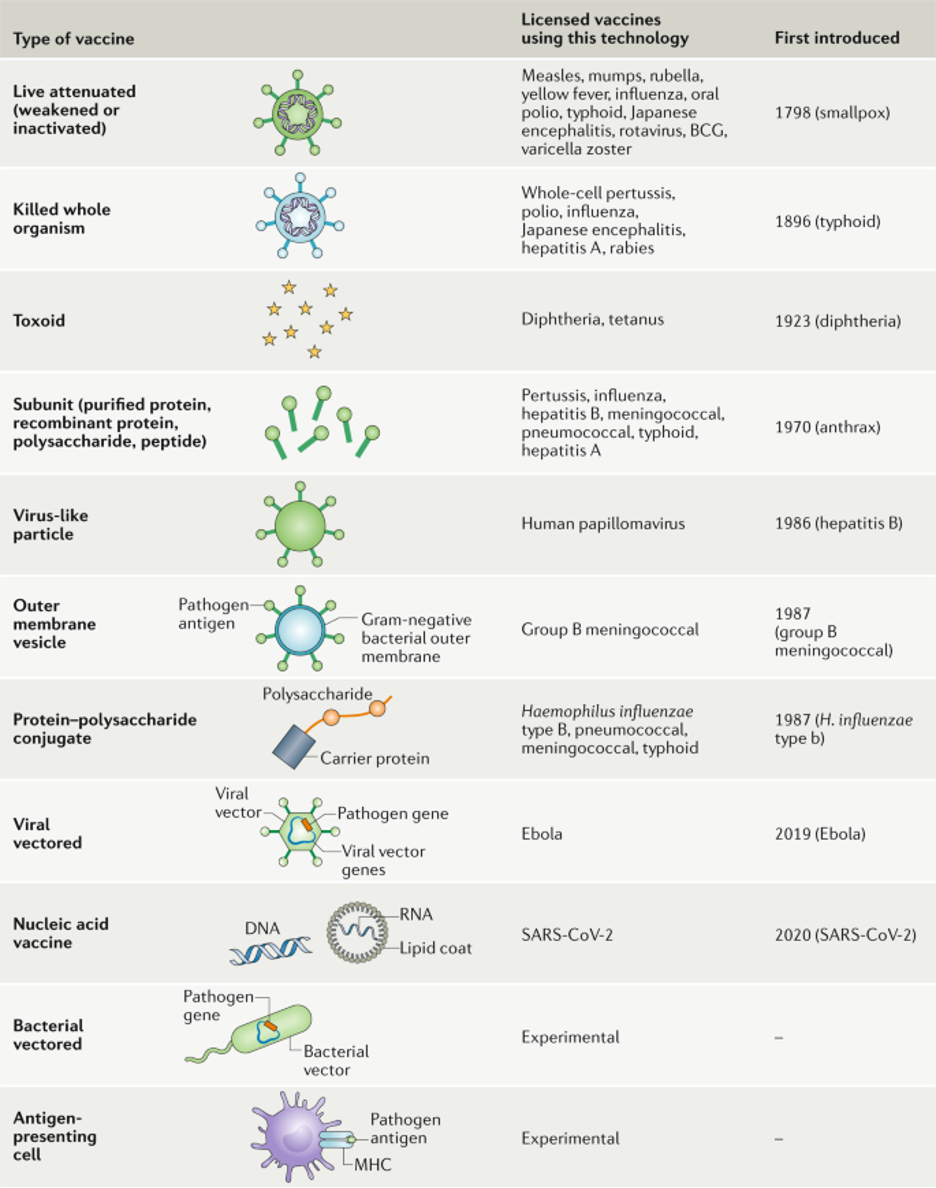
Schematic representation of different types of vaccine
Schematic representation of different types of vaccine against pathogens; the text indicates against which pathogens certain vaccines are licensed and when each type of vaccine was first introduced. BCG, Mycobacterium bovis bacillus Calmette–Guérin.
In addition to the ‘traditional’ live and non-live vaccines, several other platforms have been developed over the past few decades, including viral vectors, nucleic acid-based RNA and DNA vaccines, and virus-like particles.
The distinction between live and non-live vaccines is important. Live vaccines have the potential to replicate in an uncontrolled manner in immunocompromised individuals. By contrast, non-live vaccines pose no risk to immunocompromised individuals although they may not confer protection in those with B cell or combined immunodeficiency.
Live vaccines are developed so that, in an immunocompetent host, they replicate sufficiently to produce a strong immune response, but not so much as to cause significant disease manifestations. For example, the vaccines for measles, mumps, rubella and rotavirus, oral polio vaccine, the Mycobacterium bovis bacillus Calmette–Guérin (BCG) vaccine for TB and live attenuated influenza vaccine.
There is a trade-off between enough replication of the vaccine pathogen to induce a strong immune response and sufficient attenuation of the pathogen to avoid symptomatic disease. For this reason, some safe, live attenuated vaccines require multiple doses and induce relatively short-lived immunity (e.g. the live attenuated typhoid vaccine, Ty21a), and other live attenuated vaccines may induce some mild disease. About 5% of children will develop a rash and up to 15% fever after measles vaccination.
The antigenic component of non-live vaccines can be: killed whole organisms (whole-cell pertussis vaccine and inactivated polio vaccine), purified proteins from the organism (acellular pertussis vaccine), recombinant proteins (hepatitis B virus (HBV) vaccine) or polysaccharides (the pneumococcal vaccine against S. pneumoniae. Toxoid vaccines (for tetanus and diphtheria) are formaldehyde-inactivated protein toxins that have been purified from the pathogen.
Non-live vaccines are often combined with an adjuvant to improve their ability to induce an immune response (immunogenicity). There are only a few adjuvants that are used routinely in licensed vaccines. However, the portfolio of adjuvants is steadily expanding, with liposome-based adjuvants and oil-in-water emulsions being licensed in the past few decades. The mechanism of action of aluminium salts (alum), although extensively used as an adjuvant for more than 80 years, remains incompletely understood, but there is increasing evidence that immune responses and protection can be enhanced by the addition of newer adjuvants that provide danger signals to the innate immune system. Examples of these novel adjuvants are the oil-in-water emulsion MF59, which is used in some influenza vaccines; AS01, which is used in one of the shingles vaccines and the licensed malaria vaccine; and AS04, which is used in a vaccine against human papillomavirus (HPV).
Vaccines contain other components that function as preservatives, emulsifiers (such as polysorbate 80) or stabilizers (for example, gelatine or sorbitol). Various products used in the manufacture of vaccines could theoretically also be carried over to the final product and are included as potential trace components of a vaccine, including antibiotics, egg or yeast proteins, latex, formaldehyde and/or gluteraldehyde and acidity regulators (such as potassium or sodium salts). Except in the case of allergy to any of these components, there is no evidence of risk to human health from these trace components found in some vaccines.
Vaccines induce antibodies
The adaptive immune response is mediated by B cells that produce antibodies (humoral immunity) and by T cells (cellular immunity). All vaccines in routine use, except BCG (which is believed to induce T cell responses that prevent severe disease and innate immune responses that may inhibit infection), are thought to mainly confer protection through the induction of antibodies. There is considerable supportive evidence that various types of functional antibody are important in vaccine-induced protection, and this evidence comes from three main sources: immunodeficiency states, studies of passive protection and immunological data.
Immune response following immunization with conventional protein antigen.
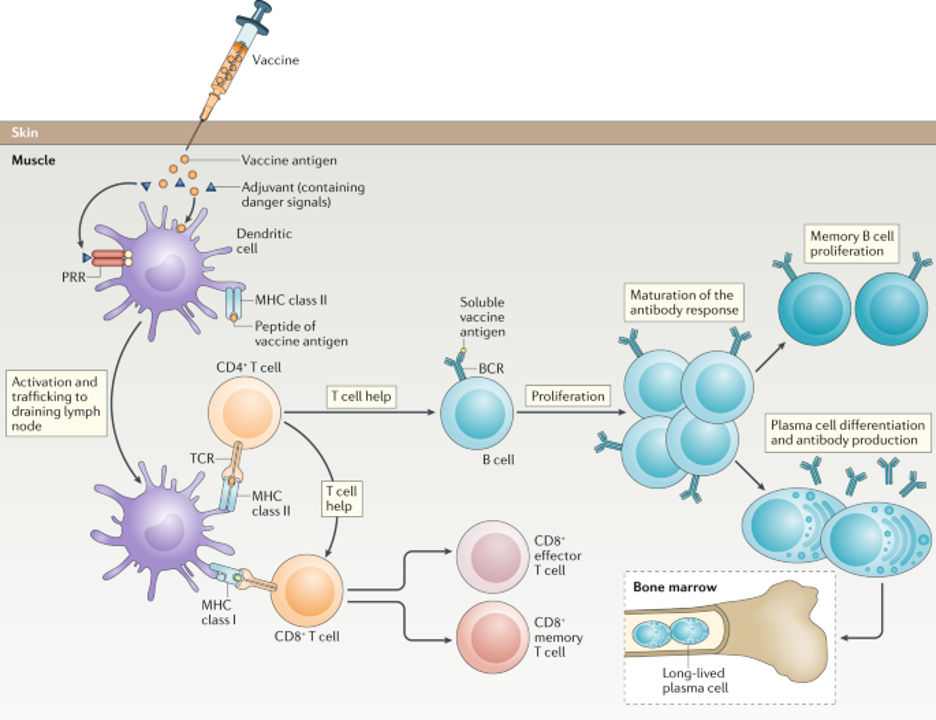
Generation of immune response to a vaccine
The vaccine is injected into muscle and the protein antigen is taken up by dendritic cells, which are activated through pattern recognition receptors (PRRs) by danger signals in the adjuvant, and then trafficked to the draining lymph node. Here, the presentation of peptides of the vaccine protein antigen by MHC molecules on the dendritic cell activates T cells through their T cell receptor (TCR). In combination with signaling by soluble antigen through the B cell receptor (BCR), the T cells drive B cell development in the lymph node. Here, the T cell-dependent B cell development results in maturation of the antibody response to increase antibody affinity and induce different antibody isotypes. The production of short-lived plasma cells, which actively secrete antibodies specific for the vaccine protein, produces a rapid rise in serum antibody levels over the next 2 weeks. Memory B cells are also produced, which mediate immune memory. Long-lived plasma cells that can continue to produce antibodies for decades travel to reside in bone marrow niches. CD8+ memory T cells can proliferate rapidly when they encounter a pathogen, and CD8+ effector T cells are important for the elimination of infected cells.
Immunodeficiency states
Individuals with some known immunological defects in antibodies or associated immune components are particularly susceptible to infection with certain pathogens, which can provide insight into the characteristics of the antibodies that are required for protection from that particular pathogen.
For example, individuals with deficiencies in the complement system are particularly susceptible to meningococcal disease caused by infection with Neisseria meningitidis21 because control of this infection depends on complement-mediated killing of bacteria, whereby complement is directed to the bacterial surface by IgG antibodies. Pneumococcal disease is particularly common in individuals with reduced splenic function (which may be congenital, resulting from trauma or associated with conditions such as sickle cell disease); S. pneumoniae bacteria that have been opsonized with antibody and complement are normally removed from the blood by phagocytes in the spleen, which are no longer present in individuals with hyposplenism. Antibody-deficient individuals are susceptible to varicella zoster virus (which causes chickenpox) and other viral infections, but, once infected, they can control the disease in the same way as an immunocompetent individual, so long as they have a normal T cell response.
Passive protection
It has been clearly established that intramuscular or intravenous infusion of exogenous antibodies can provide protection against some infections. The most obvious example is that of passive transfer of maternal antibodies across the placenta, which provides newborn infants with protection against a wide variety of pathogens, at least for a few months after birth. Maternal vaccination with pertussis, tetanus and influenza vaccines harnesses this important protective adaptation to reduce the risk of disease soon after birth and clearly demonstrates the role of antibodies in protection against these diseases. Vaccination of pregnant women against group B streptococci and respiratory syncytial virus (RSV) has not yet been shown to be effective at preventing neonatal or infant infection, but it has the potential to reduce the burden of disease in the youngest infants. Other examples include the use of specific neutralizing antibodies purified from immune donors to prevent the transmission of various viruses, including varicella zoster virus, HBV and measles virus. Individuals with inherited antibody deficiency are without defense against serious viral and bacterial infections, but regular administration of serum antibodies from an immunocompetent donor can provide almost entirely normal immune protection for the antibody-deficient individual.
Immunological data
It is now known that polysaccharide vaccines, which are made from the surface polysaccharides of invasive bacteria such as meningococci (N. meningitidis) and pneumococci (S. pneumoniae) are T cell-independent antigens, and do not induce T cell responses. Thus they must mediate their protection through antibody-dependent mechanisms. Protein–polysaccharide conjugate vaccines contain the same polysaccharides from the bacterial surface, but they are chemically conjugated to a protein carrier (mostly tetanus toxoid, or diphtheria toxoid or a mutant protein derived from it, known as CRM197).
The T cells induced by the vaccine recognize the protein carrier, a T cell-dependent antigen, and these T cells provide help to the B cells that recognize the polysaccharide, but no T cells are induced that recognize the polysaccharide and, thus, only antibody is involved in the protection induced by these vaccines. Human challenge studies have been used to demonstrate the role of antibodies in protection against malaria and typhoid.
Vaccines need T-cell help
Although most of the evidence points to antibodies being the key mediators of immunity induced by vaccination, most vaccines also induce T cell responses although the role of T cells in protection is poorly characterized, except for their role in providing help for B cell development and antibody production in lymph nodes.
From studies of individuals with inherited or acquired immunodeficiency, it is clear that T cell deficiency results in failure to control a pathogen after infection. For example, the relative suppression of T cell responses that occurs at the end of pregnancy increases the severity of infection with influenza and varicella zoster viruses.
Traditionally, T cells have been categorized as either cytotoxic (killer) T cells or helper T cells. Subtypes of T helper cells (TH cells) can be distinguished by their profiles of cytokine production. T helper 1 (TH1) cells and TH2 cells are mainly important for establishing cellular immunity and humoral immunity, respectively, although TH1 cells are also associated with generation of the IgG antibody subclasses IgG1 and IgG3. Other TH cell subtypes include TH17 cells which are important for immunity at mucosal surfaces such as the gut and lung and T follicular helper cells located in secondary lymphoid organs, which are important for the generation of high-affinity antibodies.
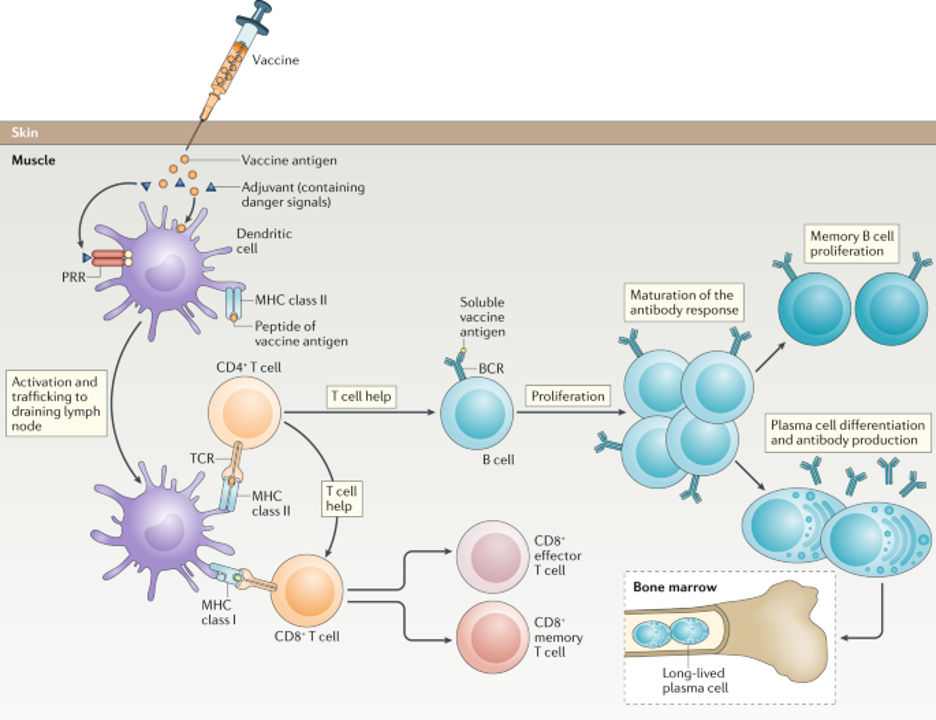
Generation of immune response to a vaccine
The evidence indicates that antibodies have the major role in prevention of infection (supported by TH cells), whereas cytotoxic T cells are required to control and clear established infection.
Vaccine induced protection
Vaccines have been developed over the past two centuries to provide direct protection of the immunized individual through the B cell-dependent and T cell-dependent mechanisms. It has become apparent that this protection is largely manifested through the production of antibodies and induction of immune memory. While vaccines are usually developed to prevent clinical manifestations of infection, some vaccines, in addition to preventing the disease, may also protect against asymptomatic infection or colonization, thereby reducing the acquisition of a pathogen and thus its onward transmission, establishing herd immunity where each dose of vaccine protects more individuals than the vaccine recipient. Some vaccines may also drive changes in responsiveness to future infections with different pathogens, so called non-specific effects, perhaps by stimulating prolonged changes in the activation state of the innate immune system. Unfortunately, the strains of SARS-CoV-2 coronavirus responsible for the Covid-19 disease appear to resist these conventional methods of control.
Immune memory
Antibody levels in the circulation wane after primary vaccination, often to a level below that required for protection. Whether immune memory can protect against a future pathogen encounter depends on the incubation time of the infection, the quality of the memory response and the level of antibodies induced by memory B cells.
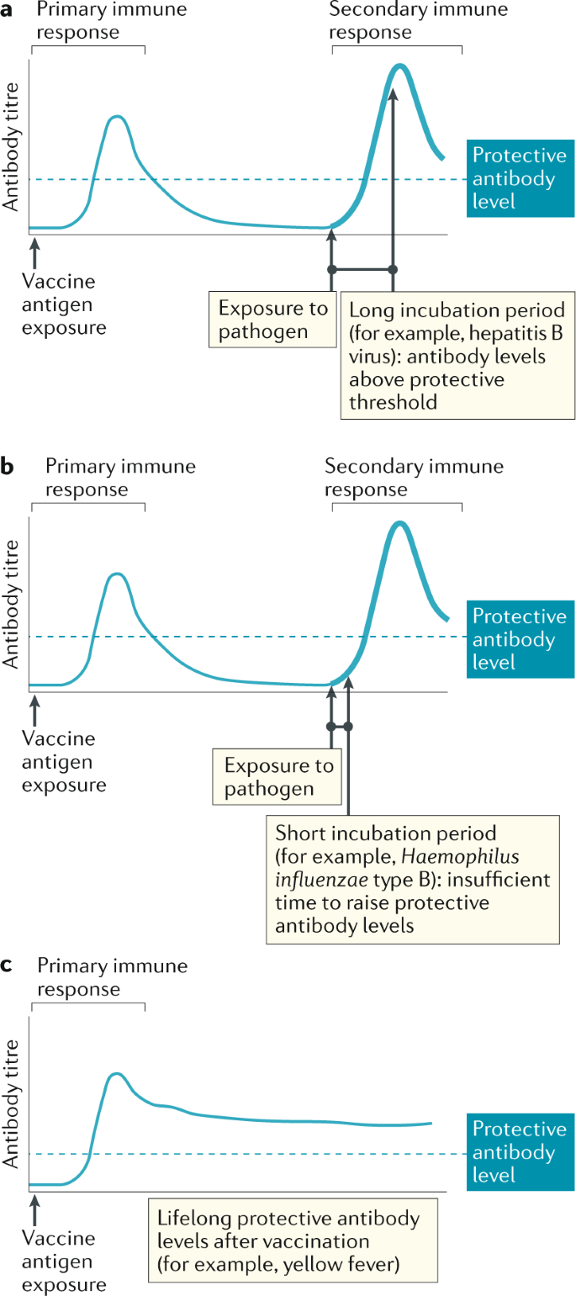
Immune memory
a | Immune memory has been shown to be sufficient for protection against pathogens when the incubation period is long enough for a new immune response to develop. The memory response may be sufficient to protect against disease if there is a long incubation period between pathogen exposure and the onset of symptoms to allow for the 3–4 days required for memory B cells to generate antibody titres above the protective threshold. For example, in the case of HBV, which has an incubation period of 6 weeks to 6 months, a vaccinated individual is usually protected following vaccination even if exposure to the virus occurs some time after vaccination and the levels of vaccine-induced antibody have already waned.
b | The memory response may not be sufficient to protect against disease if the pathogen has a short incubation period and there is rapid onset of symptoms before antibody levels have reached the protective threshold. For example, there is evidence in the case of both Haemophilus influenzae type B (Hib) and capsular group C meningococcal infection that individuals with vaccine-induced immune memory can still develop disease once their antibody levels have waned, despite mounting robust, although not rapid enough, memory responses. The waning of antibody levels varies depending on the age of the vaccine recipient being very rapid in infants as a result of the lack of bone marrow niches for B cell survival; the nature of the antigen and the number of booster doses administered. For example, the virus-like particles used in the HPV vaccine induce antibody responses that can persist for decades, whereas relatively short-term antibody responses are induced by pertussis vaccines; and the inactivated measles vaccine induces shorter-lived antibody responses than the live attenuated measles vaccine.
c | In some cases, antibody levels after primary vaccination remain above the protective threshold and can provide lifelong immunity.
For infections that are manifest soon after acquisition of the pathogen, the memory response may be insufficient to control these infections and sustained immunity for individual protection through vaccination can be difficult to achieve. One solution to this is the provision of booster doses of vaccine through childhood (as is the case, for example, for diphtheria, tetanus, pertussis and polio vaccines), in an attempt to sustain antibody levels above the protective threshold. It is known that provision of five or six doses of tetanus or diphtheria vaccine in childhood can provide lifelong protection, and so booster doses of these vaccines throughout adult life are not routine in most countries that can achieve high coverage with multiple childhood doses. For some infections which occur mostly in young children, continued boosting after the second year of life is not undertaken. For example, the invasive bacterial infections including Hib and capsular group B meningococci.
The exception is the pertussis vaccine, where the focus of vaccine programs is the prevention of disease in infancy and this is achieved both by direct vaccination of infants as well as by the vaccination of other age groups, including adolescents and pregnant women in some programs, to reduce transmission to infants and provide protection by antibody transfer across the placenta. Starting in the 1990s, many high-income countries have switched to using the acellular pertussis vaccine, which is less reactogenic than the older whole-cell pertussis vaccine that is still used in most low-income countries although it is now apparent that acellular pertussis vaccine induces a shorter duration of protection against clinical pertussis and may be less effective against bacterial transmission than is the whole-cell pertussis vaccine. Many high-income countries have observed a rise in pertussis cases since the introduction of the acellular vaccine, a phenomenon that is not observed in low-income nations using the whole-cell vaccine.
By contrast, lifelong protection seems to be the rule following a single dose with some of the live attenuated viral vaccines, such as yellow fever vaccine, although it is apparent that protection is incomplete with others. In the case of varicella zoster and measles–mumps vaccines, some breakthrough cases are described during disease outbreaks among those individuals who have previously been vaccinated, although it is unclear whether this represents a group in whom immunity has waned (and who therefore needed booster vaccination) or a group for whom the initial vaccine did not induce a successful immune response. Breakthrough cases are less likely in those individuals who have had two doses of measles–mumps–rubella vaccine or varicella zoster vaccine, and cases that do occur are usually mild, which indicates that there is some lasting immunity to the pathogen.
An illustration of the complexity of immune memory and the importance of understanding its underlying immunological mechanisms in order to improve vaccination strategies is provided by the concept of “original antigenic sin” where the immune system fails to generate an immune response against a strain of a pathogen if the host was previously exposed to a closely related strain. This has been demonstrated in several infections, including dengue and influenza. Strategies to overcome this include the use of adjuvants that stimulate innate immune responses which can induce sufficiently cross-reactive B cells and T cells that recognize different strains of the same pathogen, or the inclusion of as many strains in a vaccine as possible, the latter approach obviously being limited by the potential of new strains to emerge in the future.
Herd immunity
Vaccines cannot protect every individual in a population directly, however, if enough individuals in a population are vaccinated and , if vaccination prevents not only the development of disease but also the infection itself, then transmission of the pathogen can be interrupted and the incidence of disease can fall further than would be expected, as a result of the indirect protection of individuals who would otherwise be susceptible. This is called “herd immunity,” or more correctly “herd protection”.
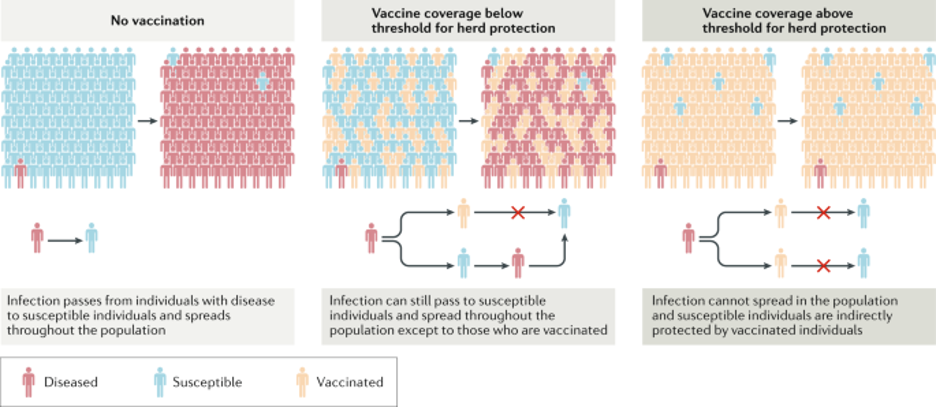
Herd immunity for highly contagious diseases
For highly transmissible pathogens, such as those causing measles or pertussis, around 95% of the population must be vaccinated to prevent disease outbreaks, but for polio, rubella, mumps or diphtheria, vaccine coverage can be ≤86%. For influenza, the threshold for herd immunity is highly variable from season to season and is also confounded by the variability in vaccine effectiveness each year. There is still no substantial credible scientific evidence that any herd immunity or herd protection exists yet for SARS-CoV-2 coronavirus or the Covid–19 disease.
Apart from tetanus vaccine, all other vaccines in the routine immunization schedule induce some degree of herd immunity which enhances population protection beyond that which could be achieved by vaccination of the individual only. Tetanus, however, is a toxin-mediated disease acquired through infection of breaks in the skin contaminated with the toxin-producing bacteria Clostridium tetani from the environment so vaccination of the community with the tetanus toxoid will not prevent an unvaccinated individual acquiring the infection if they are exposed.
Factors affecting vaccine effectiveness
Immune responses to polysaccharide and protein–polysaccharide conjugate vaccines
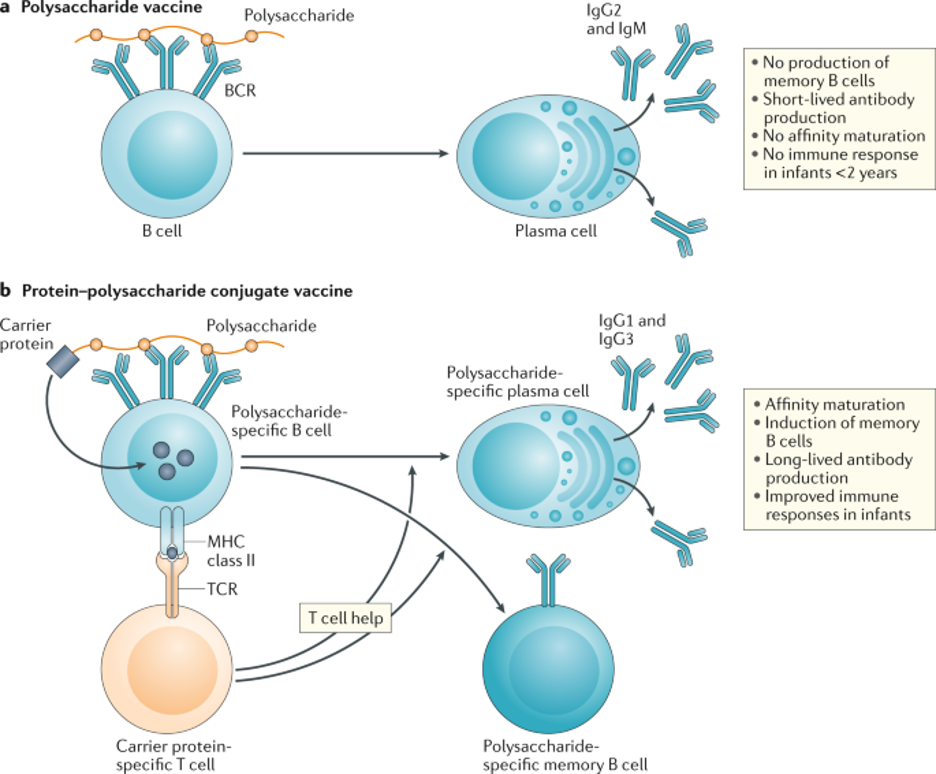
Immune responses to conjugate vaccines
a | Polysaccharide vaccines induce antibody-producing plasma cells by cross-linking the B cell receptor (BCR). However, affinity maturation of the antibody response and the induction of memory B cells do not occur.
b | Protein–polysaccharide conjugate vaccines can engage T cells that recognize the carrier protein, as well as B cells that recognize the polysaccharide. T cells provide help to B cells, leading to affinity maturation and the production of both plasma cells and memory B cells. TCR, T cell receptor.
Adapted from Pollard, A. J., Perrett, K. P. & Beverley, P. C. “Maintaining protection against invasive bacteria with protein-polysaccharide conjugate vaccines.” Nat. Rev. Immunol. 9, 213–220 (2009). This paper presents a review of the mechanism of action of polysaccharide vaccines and their role in establishing long-term protection against invasive bacteria.
Immune responses are also poor in the older populations and most of the vaccines used in older adults offer limited protection or a limited duration of protection, particularly among those older than 75 years of age. The decline in immune function with age known as immunosenescence has been well documented.
Reverse Vaccinology
Traditional and first generation vaccines are composed of live or fixed whole pathogens, while second generation vaccines include, among others, native protein antigens purified from the pathogen. Third-generation vaccines are comprised of DNA plasmids capable of expressing the sequence of the most important pathogen protein antigens in the host. During this evolution of vaccines, there has been a gain in safety but a loss of efficacy that has been compensated for with the use of adjuvants.
The latest step in the evolution of vaccine formulations is the development of epitope vaccines. Epitopes are short amino acid sequences of a protein that can induce a more direct and potent immune response, than the response induced by the whole cognate protein.
Since vaccines against parasite, bacteria, or virus infections and tumors require a cellular immune response for prevention, control, and cure, a strategy called Reverse Vaccinology (RV) was developed. The RV approach uses the information of the codon sequence contained in the DNA of the pathogen to obtain a complementary cDNA, and further translates it to obtain the sequence of the protein of interest.
The RV approach has revolutionized vaccine development by adopting computerized screening of protein sequences from the pathogen as the first step of the process, to select a subset of promising antigens, aka potential vaccine candidates (PVCs) (A below).
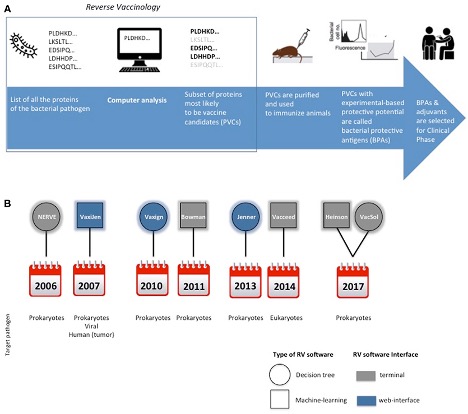
Protein subunit vaccine development
Schematically representing the main steps for protein subunit vaccines development. In the square is highlighted the Reverse Vaccinology part (A). Timeline of the delivery of RV standalone programs and their main characteristics in terms of type of software, interface and target pathogen (B).
RV offers two main advantages compared to traditional vaccine development approaches: (i) identification of candidate antigens without the need to grow the pathogen (ii) identification of any antigen independently by its purified quantity to be suitable for vaccine testing.
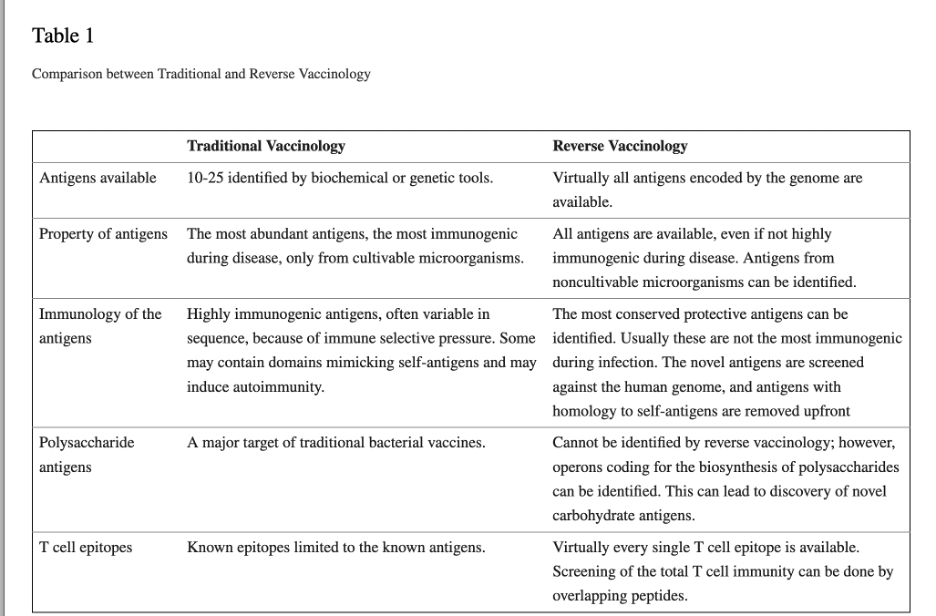
RV comparison table
Once these proteins are inside the antigen-presenting cells (APC) of the host, they are processed. The T cell epitopes are then proteolytically cleaved from the protein, and further exposed by the MHC molecules of the APC surface, to interact with the receptors of T cells. Therefore, with the knowledge of the primary sequence of the protein antigen, the epitopes can be identified by cloning the domains or smaller peptides of the protein separately and experimentally determining which one is more immunogenic, or alternatively, by screening the whole protein sequence using in silico predictions programs.
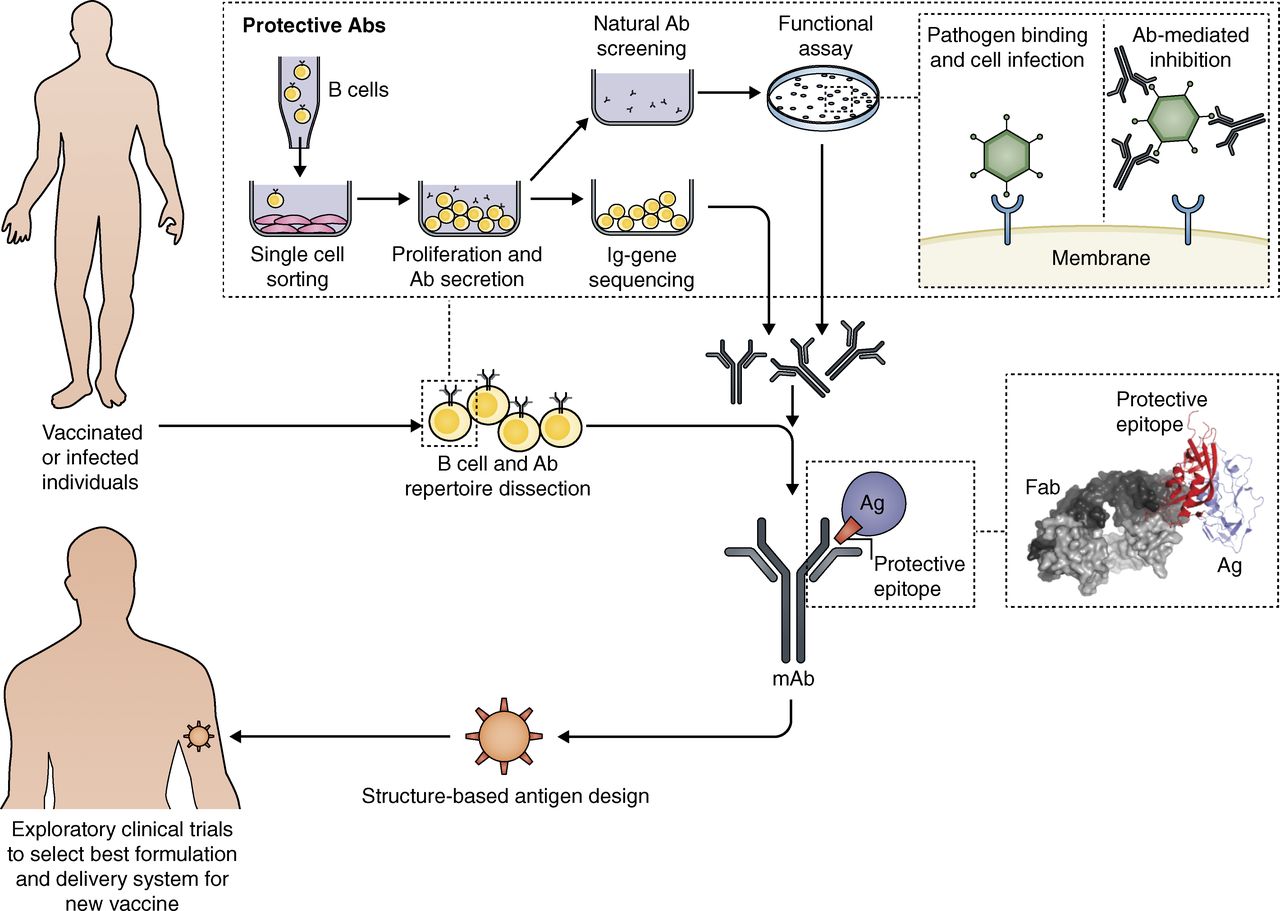
RV design process
Omicron variant effects on those already vaccinated
< style ="text-indent:2em; font-size:100%; line height:normal;">
At this stage in the SARS-CoV-2 coronavirus pandemic, there is a view that the global spread of B.1.1.529 (Omicron), through its association with a relatively milder disease phenotype and, possibly, a potential to boost vaccine immunity, may herald the transition into a new, endemic relationship.
Vaccine-mediated immune preconditioning
The case for vaccine-mediated immune preconditioning as key mediator of the attenuated phenotype is complex. A recent long-term study of healthcare workers in the United Kingdom has allowed their history of infection and vaccination to be traced precisely.
”Immune boosting by B.1.1.529 (Omicron) depends on previous SARS-CoV-2 exposure, Science 14 Jun 2022 Vol 377, Issue 6603 DOI: 10.1126/science.abq1841 https://www.science.org/doi/full/10.1126/science.abq1841?et_rid=276510521&utm_campaign=SCIeToc&af=R&et_cid=4319165&utm_medium=email&utm_content=alert&utm_source=sfmc
Researchers found some unexpected immune-damping effects caused by infection with a heterologous variant to the latest wave of infection by the Omicron/Pango lineage B.1.1.529.
Hybrid immune damping
Vaccine boosting results in distinct, imprinted patterns of hybrid immunity with different combinations of SARS-CoV-2 infection and vaccination. Immune protection is boosted by B.1.1.529 (Omicron) infection in the triple-vaccinated, previously infection-naïve individuals, but this boosting is lost with prior Wuhan Hu-1 imprinting. This “hybrid immune damping” indicates substantial subversion of immune recognition and differential modulation through immune imprinting and may be the reason why the B.1.1.529 (Omicron) wave has been characterized by breakthrough infection and frequent reinfection with relatively preserved protection against severe disease in triple-vaccinated individuals.
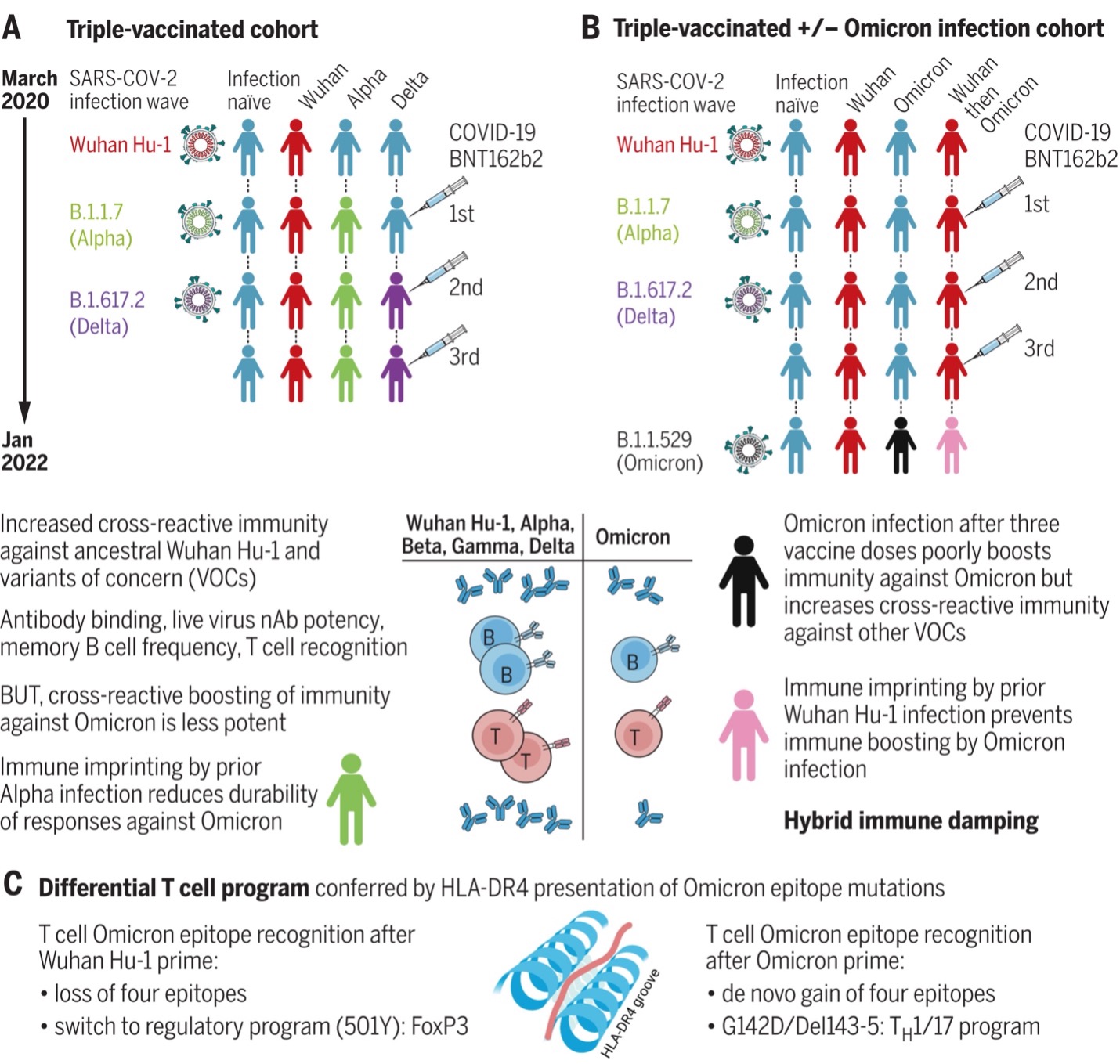
Hybrid immune damping
(A) Triple-vaccinated HCWs with different SARS-CoV-2 infection histories show boosted cross-reactive immunity against VOCs, less so against Omicron. (B) Breakthrough infection during the Omicron wave boosts cross-reactive immunity in triple-vaccinated, previously infection-naïve individuals against VOCs, less so against Omicron itself; imprinting by previous Wuhan Hu-1 infection ablates Omicron immune boosting. (C) T cell recognition of Omicron mutation sequences is linked to altered transcription.
https://www.science.org/doi/full/10.1126/science.abq1841?et_rid=276510521&utm_campaign=SCIeToc&af=R&et_cid=4319165&utm_medium=email&utm_content=alert&utm_source=sfmc
B and T cell recognition and nAb potency were boosted against previous variants of concern (VOCs), but this enhanced immunity was attenuated against B.1.1.529 (Omicron) itself. Furthermore, immune imprinting after B.1.1.7 (Alpha) infection resulted in reduced durability of antibody binding against B.1.1.529 (Omicron), and S1 RBD and whole spike VOC binding correlated poorly with live virus nAb potency.
Half of triple-vaccinated HCWs showed no T cell response to B.1.1.529 (Omicron) S1 processed antigen, and all showed reduced responses to the B.1.1.529 (Omicron) peptide pool, irrespective of SARS-CoV-2 infection history. Mapping T cell immunity in class II human leukocyte antigen transgenics showed that individual spike mutations could result in loss or gain of T cell epitope recognition, with changes to T cell effector and regulatory programs.
Triple-vaccinated, previously infection-naïve individuals infected during the B.1.1.529 (Omicron) wave showed boosted cross-reactive S1 RBD and whole spike binding, live virus nAb potency, and T cell immunity against previous VOCs but less so against B.1.1.529 (Omicron) itself.
Immune imprinting from prior Wuhan Hu-1 infection abrogated any enhanced cross-reactive antibody binding, T cell recognition, MBC frequency, or nAb potency after B.1.1.529 (Omicron) infection.